Translate this page into:
Photoacoustic Imaging: Opening New Frontiers in Medical Imaging
Address for correspondence: Keerthi S. Valluru, Department of Imaging Sciences, University of Rochester, 601 Elmwood Ave, Box 648, Rochester, NY 14642, USA. E-mail: keerthi_valluru@urmc.rochester.edu
-
Received: ,
Accepted: ,
This is an open-access article distributed under the terms of the Creative Commons Attribution License, which permits unrestricted use, distribution, and reproduction in any medium, provided the original author and source are credited.
This article was originally published by Medknow Publications & Media Pvt Ltd and was migrated to Scientific Scholar after the change of Publisher.
Abstract
In today's world, technology is advancing at an exponential rate and medical imaging is no exception. During the last hundred years, the field of medical imaging has seen a tremendous technological growth with the invention of imaging modalities including but not limited to X-ray, ultrasound, computed tomography, magnetic resonance imaging, positron emission tomography, and single-photon emission computed tomography. These tools have led to better diagnosis and improved patient care. However, each of these modalities has its advantages as well as disadvantages and none of them can reveal all the information a physician would like to have. In the last decade, a new diagnostic technology called photoacoustic imaging has evolved which is moving rapidly from the research phase to the clinical trial phase. This article outlines the basics of photoacoustic imaging and describes our hands-on experience in developing a comprehensive photoacoustic imaging system to detect tissue abnormalities.
Keywords
Acoustic lens based imaging
cancer detection
c-scan imaging
photoacoustic imaging
INTRODUCTION
Every imaging modality depicts non-invasively a specific tissue property that results from an interaction between the tissue and the modality's inherent feature. For example, X-ray and computed tomography depict the attenuation of X-rays, ultrasound depicts one of the mechanical properties (reflectivity or acoustic impedance difference) of the tissue, and positron emission tomography depicts the concentration of injected radio nucleotide in the body. Similarly, photoacoustic (PA) imaging depicts optical absorption properties of a tissue at the wavelength of interrogating laser light similar to pure optical imaging but with noticeable differences. These properties are presented below.
As the name suggests, the PA effect is a combination of both light and sound. When a laser pulse of short duration, approximately a few nanoseconds (ns), interacts with the tissue, it results in localized heating of the tissue followed by rapid thermal expansion, generating thermo-elastic stress waves. This effect is known as a PA effect and the resulting stress (ultrasound) waves are referred to as PA waves.
PA imaging is neither purely optical nor purely acoustical in nature, but a combination of the two. Pure optical imaging uses light as the input and the backscattered radiation as the output for image formation, whereas PA imaging uses light as the input source for tissue excitation but detection and image formation are achieved using ultrasound waves generated by the tissue. It keeps the best of both the optical and acoustical parts. For example, due to excessive scattering of light in turbid media, optical imaging suffers from poor penetration depths and low resolution.[1] PA imaging has better penetration depths because the incident light has to propagate in only one direction into the tissue and the ultrasound waves do not attenuate as much in the tissue as backscattered light. A good resolution in PA imaging results from the fact that the ultrasound can be focused easily and speed of propagation does not deviate much (for instance, it is ~ 1500 m/s in soft tissue) so that the wave arrival time can provide information about the depth. The tissue and its major constituents (water, oxy and de-oxy hemoglobin in blood, lipid, fat, melanin, collagen, etc) have widely varying absorption spectra[2] in the near infrared (NIR) region that not only provide abundant contrast features, but also open the door for PA imaging based spectroscopy, non-invasive tissue characterization and functional imaging. Photoacoustically generated ultrasound waves and typical echo-based ultrasound waves are similar from a detector standpoint as all that is needed to capture the signals is an ultrasound transducer. However, it is important to mention two vital characteristics of photoacoustically generated ultrasound waves that differentiate them from echo-based ultrasound waves. First, the frequency of echo-based ultrasound signal in a medical ultrasound imaging system lies within the bandwidth of the transmitted signal, whereas the frequency of the PA signal depends on the size of the biological absorber and the duration of the illuminating laser pulse. Second, unlike conventional ultrasound where the signal strength or image intensity depends on the echogenicity (ultrasound reflectivity) of the echo-generating source in the medium, the PA signal strength depends on the optical absorption coefficient of the absorber in the medium and the difference in optical absorption of the incident light dictates the image contrast, as mentioned earlier.
Absorption coefficients of tissue constituents vary with wavelength of incident light. Also, since angiogenesis is the fundamental step in the transition of a tumor from dormant to malignant state, a tumor that has blood vessels proliferated into it, interrogated at appropriate wavelengths will produce a stronger PA signal compared to the surrounding normal tissue.[3–5] The major part of a tissue is made up of water that has a lower absorption coefficient in the near infrared (NIR) region, especially in the wavelength range of 650-950 nanometers (nm), than blood and other tissue constituents.[26] If the tissue is illuminated at these wavelengths, deeper penetration of light can be achieved and the tissue can be imaged with better contrast and the tissue characteristics such as oxy and deoxy-hemoglobin concentrations, oxygen saturation, and other parameters, can be extensively studied. Hence, this wavelength range is commonly called the “biological window or therapeutic window” for tissue imaging. Since PA imaging can yield images from depths of up to a few centimeters, it provides a map of the optical absorption by tissue constituents at the interrogating laser wavelength along with the depth or location information. Therefore, the PA signal is more diagnostic in nature than the conventional ultrasound signal.
C-scan concept and acoustic lens-based photoacoustic imaging
A pulse-echo medical ultrasound system typically provides two-dimensional images of the underlying three-dimensional organ or tissue being scanned, which are technically called B-scan images. These B-scan images essentially provide depth-resolved anatomical information. As the imaging depth increases, the lateral resolution in the B-scan images gets worse and to correct for it, electronic focusing techniques are implemented in the software.[7] Similar focusing has been achieved using acoustic lens. The advantages of the acoustic lens focusing over electronic focusing are: (1) it is faster, (2) it uses zero electrical power, (3) it is inexpensive to design and implement, and (4) there are no errors in the reconstructed image due to spatial and temporal sampling of the signal. The disadvantage is that focusing is limited to a fixed depth. However, our use of lens-based focusing is different from what has been done in the past in clinical ultrasound imaging. Earlier, the focusing element was typically placed on the single element transducer, while the transducer was scanned in sectors to acquire the ultrasound image. This is time consuming. In our version, by separating the lens from the image plane, we can in principle acquire the focused image on the image plane (coronal plane) almost instantaneously with a two-dimensional sensor array. Figure 1shows our acoustic lens-based PA imaging concept. When a near-infrared laser illuminates the tissue with very short high energy pulses of nanosecond duration, it generates PA waves within the tissue if any absorbers are present. An acoustic lens will collect the waves generated in the object plane and focus them onto an ultrasound detector which is placed in the image plane [Figure 1]. An appropriately designed lens will focus the wave front from a point source in the object plane to a point in the image plane. The object plane here corresponds to a coronal plane in the tissue unlike sagittal or transverse planes imaged by a conventional ultrasound imaging system. Image plane is defined by time delay measured with respect to time t=0 when the laser illuminates the tissue. In our experience, the difference in travel time from different points in the object plane to their corresponding focus points in the image plane is usually less than the pulse duration of the PA signal. Therefore, the entire image plane can be assumed to be in focus and is said to be the conjugate image plane corresponding to the object plane defined by the delay time. More details about the lens can be found under the “PA image formation” section.
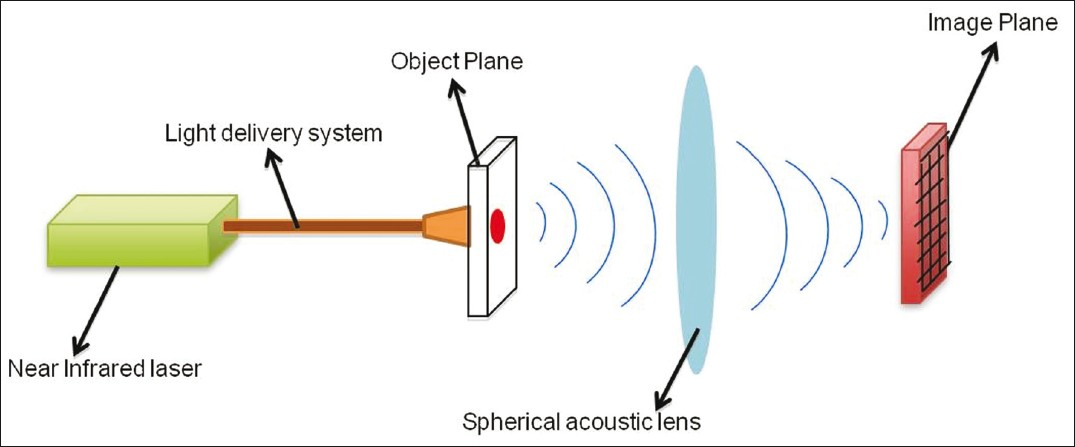
- Acoustic lens-based photoacoustic imaging. Object plane lies within the tissue and an ultrasound detector placed in the image plane will collect focused PA signals.
A detector array located in the image plane can capture this energy as a focused image that represents a thin coronal slice in the object. Images of these coronal planes are referred to as C-scan images and represent laser absorption by different regions of the tissue that are intersected by that plane, much like CT scan images. The photoacoustically generated ultrasound waves travel with finite speed (~1500 m/s) in soft tissue. Therefore, a pragmatic way to capture focused signal from a predetermined coronal plane is to time gate it when it is detected in the image plane. The assumption here is that all waves from the predetermined coronal plane arrive at the image plane at the same time.
Photoacoustic imaging prototype system for in vitro studies
As a first step toward designing an in vivo PA imaging system, we have developed a prototype PA in vitro imaging system to detect any malignancies present in an excised tissue as illustrated in Figure 2. The system design was optimized to generate focused coronal plane (C-scan) images using acoustic lens technology. In principle, C-scan images are nothing but two-dimensional (2D) images produced by spatially sampling the PA signal amplitude at a fixed time while the ultrasound sensor is raster-scanned over some surface.

-
In vitrophotoacoustic imaging probe.
The in vitro prototype system comprise of the following components: A fiber optic cable for laser delivery, a sample holder to hold the excised tissue, a PA camera that is water-sealed with acoustic lens, and the ultrasound detector. A near infrared laser beam is delivered from the bottom of the tissue sample holder, which can hold a tissue up to 4 cm thick. The laser beam has a diameter of 5 mm with a repetition rate of 10 Hz and pulse duration of 10 ns and is delivered onto the tissue via a fiber optic cable. At the sample surface, laser energy intensity is ~ 20 mJ/cm2, which is well below laser safety limits.[8]
We have designed the front-end and back-end electronics to acquire 16-channel signals simultaneously at each laser firing; details of which are given in the subsequent sections. The entire tissue is scanned by the laser beam and the camera in tandem using linear actuators. Typical time taken for imaging a 4 × 4 cm area of an excised specimen with this system is about 7 min, the delay being mainly due to the hardware limitation posed by the linear actuator speed and stabilization time. Once the PA images are acquired, the PA camera head along with the laser delivery system is replaced by a portable ultrasound array head that captures ultrasound images that can be correlated and registered with the PA images.
Photoacoustic image formation
The ultrasound transducer array has 16 elements, each of size 0.5 mm × 1.0 mm, pitch 0.7 mm, and the center frequency of 5 MHz with 60% bandwidth. The sensor element size was chosen such that the reception angular profile of the edge elements, subtended at the lens, was within 20% of what was for the center element. The pitch was chosen to minimize any aliasing due to spatial sampling of the image plane. The acoustic lens design was accomplished using OSLO (Lambda Research Corporation, Littleton, MA, USA) ray tracing software simulation program and optimized for wavelength of 0.3 mm, corresponding to 5 MHz. The lens has a focal length (f) of 39.8 mm and a diameter of 32 mm corresponding to an active f-number of 1.2. The lens design was optimized to achieve a magnification of 1 when both the lens-to-object plane and the lens-to-image plane distances are twice the focal length of the lens. Hence, in our in vitro prototype, the transducer array was fixed at 79.6 (2f) mm from the lens in the image plane and the ultrasound waves generated from any absorbers in the object plane that are 79.6 mm from the lens will be in sharp focus and are imaged with a magnification of 1. The lens has a depth of field of approximately 5 mm on either side of the focal plane. The ultrasound sensor array located in the image plane acquires focused PA signals, much like optical image formation.
To perform C-scan imaging, the PA camera is mounted on a holder that is controlled by two linear actuators (Zaber Technologies Inc., Vancouver, British Columbia, Canada) and a raster scan is performed in the image plane to scan a 2D area by collecting PA signals at each pixel location. The PA signals acquired over all 16 channels at each linear actuator location are fed simultaneously to the custom-built back end electronics module that consists of a variable gain amplifier and a digitizer to generate PA B-scan images followed by an ultrasound scan. Once the scanning is completed with both PA and ultrasound systems, the acquired PA images are fused with ultrasound images to illustrate anatomical as well as physiological information in a single image.
Photoacoustic data acquisition and image display
A 16-channel simultaneous data acquisition (DAQ) system was custom-designed to be used with the PA imaging system whose layout is shown in Figure 3. The entire electronics can be categorized into four modules: (1) amplification of a raw PA signal; (2) sampling/digitization of an amplified PA signal; (3) dual axis stepper motor movement for C-scan imaging; and (4) a host computer with LabView (National Instruments, Austin, TX, USA) controlling the first three modules and to display real-time B-scan images.
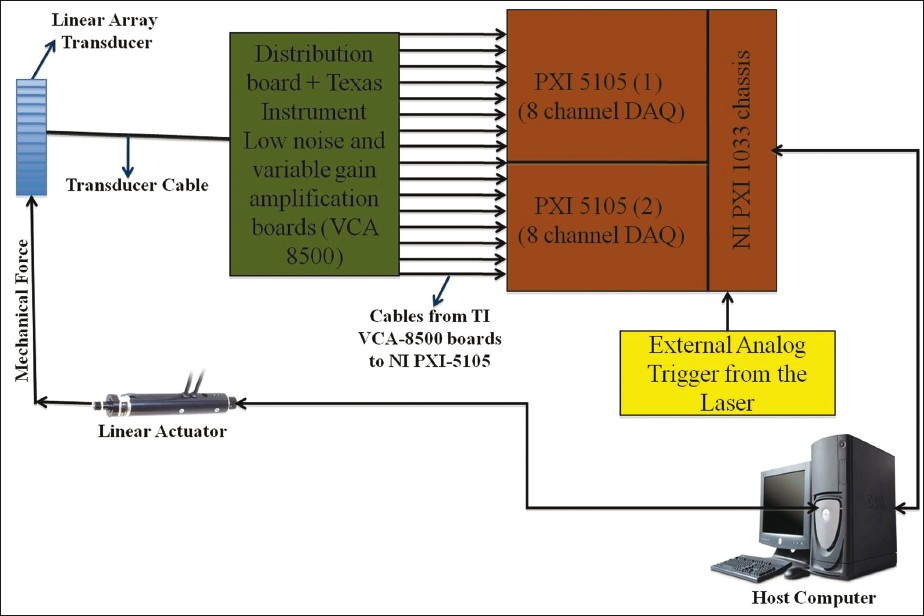
- 16-channel simultaneous data acquisition system.
PA signals acquired by the linear array transducer are tapped using a 16-channel custom-fabricated distribution board and are amplified using two 8-channel VCA8500EVM (Texas Instruments, Dallas, TX, USA) variable gain amplifiers. The variable gain amplifiers (VGAs) have a maximum amplification gain of 50 dB (a pre-amplification gain of 20 dB + selectable post gain amplification of 20, 25, 27, or 30 dB) per channel and provide the user a selection of 10 MHz or 15 MHz low pass filtering. All the 16 channels of the two VGAs are simultaneously controlled by the computer using a graphical user interface (GUI) and hence the gain can be changed on-the-fly as well.
The 16-channel amplified PA signal data is then simultaneously fed to two 8-channel PXI-5105 (National Instruments, Austin, TX, USA) digitizer boards, which are housed in an external chassis, at each laser firing. A digital trigger is provided by the laser to chassis, during each laser firing, for synchronization. This is essential for simultaneous PA image acquisition. The PXI-5105 digitizer board has 12-bit resolution and 60 MHz sampling rate with a 16 MB onboard memory buffer which allows storing the digitized PA signal samples onboard before transfer to the host computer using MXI interface.
As mentioned earlier, the total acquisition time is largely limited by the hardware specifications of the actuators; their maximum speed being only 4 mm/s, stabilization time of at least 250 ms, and a resolution of 0.1 μm. The actuators are moved by 1 mm increments along the y-axis to cover 40 mm and by 11.2 mm increments (16 × 0.7 mm) along the x-axis direction to cover 44.8 mm to scan an area of 4 cm × 4 cm. The entire scanning mechanism including DAQ and linear actuator movement is controlled by the host computer using LABVIEW. The acquired PA B-scan images are transmitted to the host computer and processed further in MATLAB environment to reconstruct C-scan images and to achieve PA/ultrasound image fusion. In vitro experiments are conducted on excised tissue samples with this system and the results obtained are presented in the following section.
RESULTS AND DISCUSSION
With the in vitro prototype system, we have performed scanning of (1) lamb kidney with a 0.7 mm lead pencil embedded 1 cm deep inside and (2) fresh lamb kidney with no external objects. Since the excised tissue is deprived of any blood within, a lead pencil was inserted into the excised kidney since the PA signal characteristics of lead are known to us by first-hand experience. The lead pencil generates strong PA signal when irradiated with 1064 nm laser. Once the PA images are acquired by the in vitro PA imaging system at a 1064 nm laser wavelength, a 40 MHz ultrasound scanner was used to acquire ultrasound images. Figure 4a shows the photograph of a 2 cm thick lamb kidney with a lead pencil of diameter 0.7 mm embedded approximately 1 cm below the top surface of the kidney. Figures 4b to 4h show the PA C-scan slices in depths varying from the anterior section to the posterior section of the kidney. Each PA C-scan slice is 0.3 mm in thickness. The lead pencil is approximately 3 mm away from the lens-focal plane and yet it is clearly reproduced in these PA C-scan images with high resolution and a magnification of 1. Figure 4i shows the ultrasound image of the lamb kidney shown in Figure 4a; Figure 4j shows the cumulative PA image obtained by adding images shown in Figures 4b to 4h. From Figure 4i, it can be noted that the area covered in the ultrasound image is only 1 cm × 1 cm, whereas the area covered in the PA image shown in Figure 4j is 4 cm × 4 cm. So, in order to achieve image co-registration, a portion of the PA image shown in the square box that covers only 1 cm × 1 cm area is cropped and then fused with the ultrasound image shown in Figure 4i to obtain an image as shown in Figure 4k.
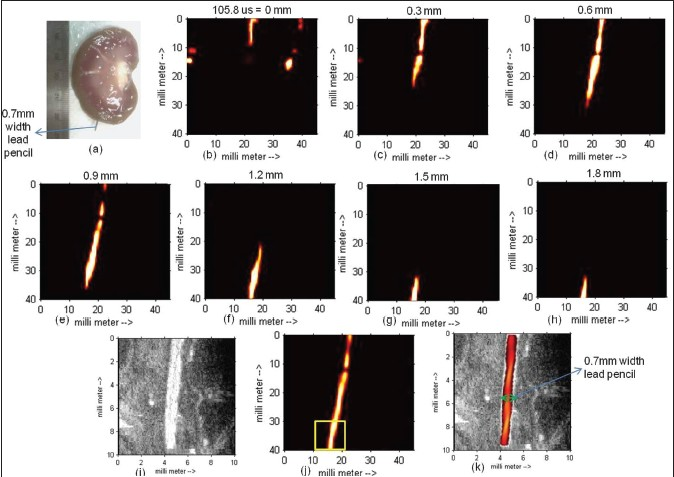
- Photoacoustic imaging of the lead pencil embedded within the lamb kidney. (a) A photograph of the lamb kidney with a lead pencil (arrow) embedded within it. (b) – (h) The 0.3 mm thick c-scan slice traversing from the anterior to posterior plane of the lamb kidney. (i) Sonogram of the lamb kidney with embedded lead pencil acquired with a 40 MHz probe. (j) PA image of lead pencil embedded within the lamb kidney by adding (b)-(h). (k) Fused ultrasound and PA image by combining (i) with a portion of PA image in (j) shown in a square box.
The second experiment was performed to detect any intrinsic PA signal from the kidney without adding any external absorbing objects to evaluate the performance of our system in detecting in vivo abnormalities. Figure 5a shows the photograph of the lamb kidney. Figures 5b and 5c show two PA coronal images at a distance of 1.5 and 1.8 mm from the anterior surface of the kidney specimen. The bright spots in Figures 5b and 5c are approximately coming from the red-colored square area indicated on the anterior surface of the kidney in Figure 5a.
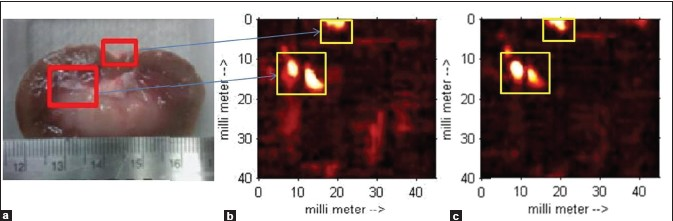
- Photoacoustic images of the lamb kidney. (a) Photograph of the lamb kidney. (b) and (c) The PA images of the kidney obtained in the coronal plane from the anterior to posterior direction at an interval of 0.3 mm. The bright spots in the yellow-colored boxes of (b), and (c) the PA signal obtained from the area within the red boxes as shown in figure (a)
We have noticed that the PA signals from the tissue are typically 10 to 100 times smaller compared to the signal from a lead pencil. Nevertheless, our system was capable of displaying PA images with low signal strength as well. This experiment illustrates the strength of PA imaging to perform in vivo imaging. To improve the strength of this intrinsic PA signal further so as to display the images with a higher contrast, an additional 20 dB amplification of PA signal from the tissue might be needed.
CONCLUSION
We believe that PA phenomenon has great promise for medical imaging in the area of early detection of cancer, guiding biopsy, imaging with targeted nano-particles, application of spectroscopy, and blood oxygen level determination, just to name a few. It is a safe and non-invasive or at worst, minimally invasive modality. We believe the trend is set for the development of more clinical PA imaging systems and more clinical trials are required that will be able to demonstrate the potential of PA phenomenon. We have presented in this paper for the first time an innovative acoustic lens-based focusing of a PA signal and a C-scan image generation concept. Using this concept, we have described our experience in designing and fabricating a prototype PA imaging system for imaging an excised tissue. Our current imaging system has a resolution of 0.7 mm in the coronal plane and can generate C-scan slices that are 0.1 mm apart. The advantage of our technology is that the need for software based image reconstruction is eliminated, it can image coronal planes along with sagittal or transverse planes and has better signal to noise ratio. Implementation of this technology into an in vivo probe is currently in progress at our research facility.
Source of Support: Nil
Conflict of Interest: None declared.
Available FREE in open access from: http://www.clinicalimagingscience.org/text.asp?2011/1/1/24/80522
REFERENCES
- Measurement of the penetration depths of red and near infrared light in human ex vivo tissues. J Photochem Photobiol B. 2000;57:90-3.
- [Google Scholar]
- Mechanics of pulsed laser ablation of biological tissues. Chem Rev. 2003;103:577-644.
- [Google Scholar]
- Basics and clinical applications of photoacoustic imaging. Ultrasound Clin. 2009;4:403-29.
- [Google Scholar]
- Handbook of optical biomedical diagnostics. Washington: SPIE-The International Society for Optical Engineering; 2002.
- [Google Scholar]
- A Clearer vision for in vivo imaging. In: Nature Biotechnol. Vol 19. 2001. p. :316-7.
- [Google Scholar]
- Ultrasound imaging in encyclopedia of imaging science and technology. New Jersey: John Wiley and Sons; 2002. p. :1412-35.
- [Google Scholar]
- American National Standard for Safe Use of Lasers. In: Laser Institute of America. New York: ANSI Z136.1; 2000.
- [Google Scholar]